Volume 11 - Year 2024 - Pages 322-334
DOI: 10.11159/jffhmt.2024.032
Novel Composites: Synergistic Effects of Graphene Oxide, Conducting Polymers and Metal Oxides in Supercapacitor Electrodes
Paramjit Singh1*, Rashmi Saini2, Deepika2, Rajesh Kumar2, Avtar Singh3,4
1Department of Physics, Gujranwala Guru Nanak Khalsa College, Civil Lines, Ludhiana- 141001, Punjab, India.
2USBAS, Guru Gobind Singh Indraprastha University, New Delhi- 110078, India.
3Research and Development, Molekule Group Inc, 3802 Spectrum BLVD., Tampa, Florida- 33612, USA.
4Department of Chemistry, Sri Guru Teg Bahadur Khalsa College, Anandpur Sahib-140118, Punjab, India.
Abstract - The development of high-efficiency electrode materials for supercapacitors (SCs) has garnered significant attention, with conducting polymers (CPs) emerging as promising candidates due to their high porosity, cost-effectiveness, ease of synthesis, and tunable electrical conductivity. However, CPs often face limitations in terms of cycle stability and energy density. Recent research has focused on the synergistic integration of CPs with metal oxides (MOs) and carbon-based materials, forming composite electrodes that exhibit enhanced conductivity, mechanical durability, and improved electrochemical performance. This review highlights the novel approach of combining CPs with MOs and graphene derivatives to address these limitations, leading to superior energy storage capabilities. By presenting an overview of recent advancements in this field, we aim to elucidate the mechanisms underlying these synergistic interactions and their impact on electrode performance. This article underscores the potential for innovation in the design of next-generation supercapacitors, paving the way for more efficient and durable energy storage solutions.
Keywords: Supercapacitor, polyaniline, metal oxides, graphene, nanocomposites
© Copyright 2024 Authors - This is an Open Access article published under the Creative Commons Attribution License terms. Unrestricted use, distribution, and reproduction in any medium are permitted, provided the original work is properly cited.
Date Received: 2024-03-07
Date Revised: 2024-07-29
Date Accepted: 2024-09-04
Date Published: 2024-10-01
*Corresponding authors: psd1985@gmail.com (Paramjit Singh)
Abbreviations: Conducting polymers (CPs), supercapacitors (SCs), surface area (@SA), specific capacitance (@spc), reduced graphene oxide (R@GO),electrical conductivity (@EC), metal oxides (MOs), transition metal oxides (T@MOs), metal-organic frameworks (@MOFs), pseudocapacitor (PSC), Asymmetric SCs (ASC), graphene (GP), polyaniline (P@Ani), polypyrrole (P@Py), carbon nanotube (CNT), current density (@CD), energy density (@ED), power density (@PD)
1. Introduction
Among the most well-known types of charge storage devices made from sustainable energy sources nowadays are supercapacitors (SCs). Their exceptional power and energy densities make them high-efficiency energy storage devices. SCs are being used in load-levelling systems for heavy-duty automobiles, hybrid platforms for buses, pickups, light rail, and electric cars, as well as load-levelling intermittent renewable energy sources. Compared to conventional solid-state electrolytic capacitors, commercially available SCs can achieve approximately ~5 Wh kg−1, a substantially higher energy density (@ED); nevertheless, this is still much less than that of batteries (which can reach up to ~200 Wh kg−1) and fuel cells (which can reach up to ~350 Wh kg−1) [1]. Both active (such as electrodes and electrolytes) and passive (such as binders, separators and current collectors) components are necessary for a SC to perform. Furthermore, a separator functions as an ion-permeable barrier between electrodes and electrolytes, facilitating ion flow and providing electrical insulation to avoid short circuiting [2].
The performance of the SC, which is crucial for charge retention, is greatly influenced by the selection of electrode materials. Lately, scientists have focused on developing nanomaterials to enhance the capacitive performance of SCs [3]. The utilisation of fibres as templates has yielded advantages in theory and practice due to their higher specific surface area (@SA), which speeds up the diffusion of electrolytes and ions. Moreover, the electrochemical behaviour of the fiber-based materials is believed to be much improved by the inclusion of redox-active components, such as conducting polymers (CPs) and transition metal oxides (T@MOs), to the fibres [4]. The three key components that determine a SC's performance are its constituent materials' accessible @SA, porosity and conductivity [5]. The electrode's @SA is the main factor that determines how effective it is, which means the higher charge storage is made possible by a bigger @SA. The porosity further increases electrochemical performance and the conductivity enhances @PD [6, 7].
The materials that can be utilised to make these electrodes include conductive polymers (CPs), metal hydroxides, metal oxides (MOs), and porous carbon [8]. Because of its large @SA and low cost, the carbon material possesses a notable @PD. Its ability to store energy in electric double-layer capacitors (EDLCs) is widely known [9]. Moreover, MOs' characteristics facilitate ion transport, which is necessary for the electrochemical operation of SCs [10]. The most often used MOs in SC electrodes are MnO2, RuO2, NiO, SnO2, IrO2, Fe3O4, V2O5, Co2O3, and MoO2 [11].
Many materials, including conducting polymers (CPs), metal hydroxides, metal oxides (MOs) and porous carbon, can be used to create these electrodes [8]. The carbon material has a significant @PD because of its huge @SA and inexpensive cost. It is well recognised for its capacity to store energy in electric double-layer capacitors (EDLCs) [9]. Furthermore, the electrochemical functioning of SCs depends on ion transport, which is supported by the properties of MOs [10]. In SC electrodes, MnO2, SnO2, IrO2, RuO2, Fe3O4, V2O5, NiO, Co2O3 and MoO2 are the most often utilised MOs [11].
Nanostructured electrodes can increase SC efficiency and 3D structured designs can produce a better @ED [12]. Temperature and current density (@CD) have a major influence on the operating dynamics of SCs and thus affect their performance [13, 1]. The optimized and controlled synthesis methods of the nanostructured composites of reduced graphene oxide (R@GO) with MOs/polymers can further yield the required key features stated above. In this review we shall discuss the synthesis techniques and the different composites of CPs (especially focussed upon polyaniline (P@Ani)), MOs and graphene (GP).
2. Classification of SCs:
A draft SC, also known as an ultracapacitor, is generally constructed using a duo of electrodes (positive and negative) immersed in an electrolyte solution, with a separator dividing the electrodes [14]. Based on the charge storage mechanism, SCs can be classified into three distinct categories: (a) Electric double-layer capacitor (EDLC), (b) Pseudocapacitor (PSC) and (c) Asymmetric SCs (ASC) or hybrid SCs.
Pseudocapacitive electrode materials store charge by Faradaic processes that occur on or near the active material's surface. When an electrode material has capacitive electrochemical characteristics yet stores charge by charge-transfer Faradaic reactions across the double layer, it is said to exhibit pseudo capacitance [15]. A hybrid capacitor, also known as an asymmetrical SC, is created when two electrodes have two different charge-storage mechanisms: one capacitive and one battery-type Faradaic [16].
In EDLC mechanism, an electronically conducting electrode is submerged in an ion-conductive electrolyte solution, as a result of which, a double layer of charge is spontaneously created at the electrode-electrolyte interface. This electrostatic charge adsorption (at the electrode-electrolyte contact) stores the charge physically which is a ‘non- Faradic process’ (i.e. unlike a pseudo-capacitor, no charge transfer takes place between the electrode and electrolyte surfaces). The specific capacitance (@spc) of an EDLC is influenced by the charge adsorption property, which is mostly reliant on the surface characteristics of the carbon materials and the accessible @SA of the electrode materials [1]. In simple words, more is the @SA, more charge is adsorbed and hence more is the @spc of an EDLC.
Thus, it is preferred to use carbon materials with high @SA, adequate electrochemical stabilities, and a wide porosity to electrolyte ions, activated carbon, carbon nanomaterials (such GP and CNTs), carbon aerogels and templated carbon are a few examples of these materials [1]. GP distinguishes itself among other carbon materials due to high @SA, high electrical conductivity (@EC) and high @spc. It also progressively supplants CNT, which necessitate an intricate and expensive purification process to improve their low @ED. This transition is driven by GP's cost-efficiency and superior performance in SCs, as noted by Strauss et al. [17]. Additively, GP is not only one of the thinnest materials but also markedly stronger than its counterparts.
3. Conducting Polymers (CPs)
The polymers are broadly classified into two categories including conducting and non- CPs. The polymers are intensively researched due to their toughness, flexibility, heat resistance, radiation sensitiveness, cost- effectiveness, durability etc [18-22]. The highly distinctive property of CPs being organic materials with @EC has made them an intriguing subject of study. They have drawn the interest of numerous researchers working on edge technology applications since their discovery. Poly(sulfurnitride) or (SN)x was the first material to be developed historically for these materials. Among the others, polyacetylene, N- or S- poly-heterocycles, P@Ani and polypyrrole (P@Py) are most researched [23]. We shall focus our discussion upon P@Ani and P@Py only.
3.1 Polyaniline (P@Ani)
Many studies have been conducted on polyaniline (C6H5N) as a material for SC and battery electrodes. The ease of synthesis and remarkable environmental stability of P@Ani allow for easy doping with different acids and dopants. Furthermore, its @EC in the doped state with a Li dopant can be readily adjusted, ranging from approximately ~0.1 to ~5 S/cm [24, 25]. However, a notable drawback of P@Ani is its requirement for a proton to achieve adequate charging and discharging, requiring the employment of an acidic solution, a protic ionic liquid, or a protic solvent [26]. The molar mass of P@Ani is ~91.11 g/mol and its molecular structure is given in Fig. 1.
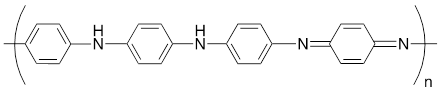
During the polymerization process of aniline monomer, P@Ani can exist in three distinct oxidation states: fully reduced leucoemeraldine base (colorless/white), partially oxidized emeraldine base (green in its salt form and blue in its base form) and fully oxidized pernigraniline base (blue/violet) [27]. Fig. 2 describes the chemical structures of all these oxidised states [28].
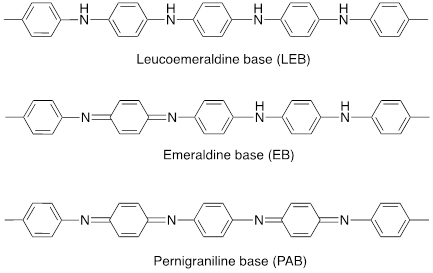
Emeraldine, which demonstrates the utmost stability and conductivity among the three states, poses difficulties in processing due to its limited solubility. The main causes of this restriction are the hydrogen bonding interactions between neighbouring chains and the rigid polymer backbone. Additionally, the practical applications of P@Ani are restricted by the instability of the emeraldine base form at elevated temperatures utilized in melt processing [30]. Depending on the particular synthesis method used, P@Ani's conductivity can be selectively adjusted by submerging the emeraldine base state in an aqueous acidic solution containing picric, phosphoric, or camphor sulfonic acid [31]. Pernigraniline and emeraldine may occur as either salts or bases [32, 33].
P@Ani has a far higher capacitance than alternative CPs because of its reversible redox reaction, which allows one electron to be removed for every two monomer units, whereas P@Py only removes one electron for every three monomer units. P@Ani's capacity can be increased by taking into account a variety of parameters, including the thickness of the electrode, the amount and type of binders and additives, and the shape of the polymers [34-36, 29]. P@Ani has many advantageous qualities when used in SC devices, including strong electroactivity, high doping levels, good stability, and a high @spc in acidic environments [37]. Applications for P@Ani include solar cells, fuel cells, SCs, lithium batteries, flexible electrodes, coatings resistant to corrosion, removal of water pollutants, screen printing, and sensors [38–46, 29]. P@Ani demonstrates remarkable adaptability in various fields owing to its tuneable electrical and electrochemical properties through doping and de- doping processes, as well as its diverse nano- and microstructures. Its reversible redox behaviour enables its application in SCs, gas sensors, pH sensors and fuel cells [47].
3.2 Polypyrrole (P@Py)
P@Py is heterocyclic, positively charged and nitrogen-containing in its oxidised state but it loses both its conductivity and charge after overoxidation [48- 50]. This CP is also biocompatible, nontoxic, and electroactive in aqueous and organic electrolyte solutions [51- 53].
The conductive nature of P@Py is attributed to the presence of alternating single and double bonds in its structure, which leads to electron density delocalization [54]. Doping further enhances the conductivity of P@Py by oxidizing the polymer and removing π-electrons, resulting in a structural transformation from an aromatic to a quinoid form [54]. This process creates a polaron, as depicted in the mechanism shown in Fig. 3. In both academic studies and practical applications, p-doping is more commonly employed, as negatively charged carriers in n-doping exhibit lower stability compared to their positively charged counterparts [55]. A doubly charged bipolaron is formed when further oxidation removes a second electron from the P@Py chain (Fig. 3). After being doped, P@Py changes into an ionic complex that includes newly added cations and counterions [54, 55].
Similar to P@Ani, P@Py is used in many other domains, such as drug delivery systems, electrodes, nanocomposites, gas sensors, biosensors, SCs, protective clothes, anticorrosion coatings, actuators, and adsorbents for the removal of dyes and heavy metals [56–59, 50].
A major disadvantage is the restricted cycling stability of CPs during charge-discharge cycles, which is caused by the polymer backbone's expansion and contraction [60–62]. In order to overcome these difficulties, high @PD, electrochemical stability, quick charge-discharge kinetics, and low self-discharge can be attained by adding nanofillers to CPs [63]. In order to accomplish this goal, composite materials must be optimised through doping concentration adjustments, thickness and dimension controls for nanofiller, uniform nanofilm distribution, and polymer/CNT/GP ratio optimisation, which includes regulating the size and dispersion of carbon materials within the polymer matrix [64–67].
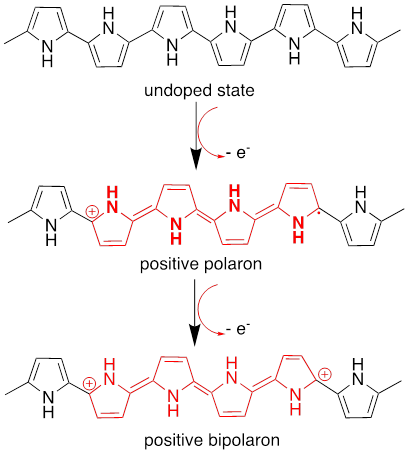
4. Composite fabrication techniques
The following methods are employed for the synthesis of GP composites with polymers.
4.1 In-situ Polymerization
Single-step and two-step processes are the two forms of in situ polymerization procedures. The two steps of the two-step process are pre-polymerization and polymerization; pre-polymerization is not required for the single-step method. The objectives of the study decide about the type of step to be followed. For instance, the single step method might be used when quick production is desired. GO sheets are first dispersed in polar solvents using ultrasonication, then the chosen monomer is added to create a homogeneous solution. This process is known as in-situ or dispersion polymerization (as shown in Fig. 4). To polymerize the monomer over the GO sheets in this solution, oxidising agents are included. This procedure is one of the most popular ways to create different morphologies of nanocomposites for increased energy storage capability. Nevertheless, this method takes longer than electrochemical deposition techniques.
4.2 Electro Polymerization
It is a widely used technique for creating composites of GP and CP. A polymer is created and applied to the surface of a solid electrode material or nanomaterial during this process. A cationic radical is produced by the monomer's oxidation on the solid electrode material, which triggers the electrochemical polymerization process. Electrochemical polymerization has two subtypes: cathodic and anodic. Aniline, thiophenes and pyrrole are just a few examples of electroactive monomers that can be electrochemically polymerized when there are inorganic nanoparticles, clusters, or metal-organic frameworks (@MOFs) present. The electrode surface receives the resultant hybrid materials [68]. Electro-polymerization can be performed on various substrates and the resulting deposited composites can be directly used as electrodes for SC applications. This method offers the advantage of simplicity and enables the generation of a polymer matrix in a relatively short time through a single-step polymerization process. Various techniques, including cyclic voltammetry, chronoamperometry and chronopotentiometry, can be employed to monitor and measure the electro-polymerization process. Typically, an electro-polymerization setup consists of three electrodes: a substrate (acting as the working electrode), a counter electrode and a reference electrode.
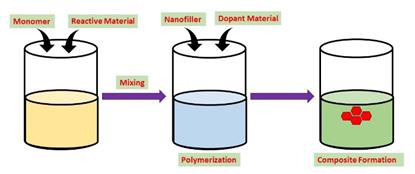
4.3 Interfacial Polymerization
Unlike in-situ polymerization, this process leads to the formation of two distinct, non-homogeneous layers that merge at their interface during the polymerization process. It occurs when monomers with different functionalities are dispersed in separate phases. Typically, this type of polymerization occurs at a liquid-liquid interface, although it can also take place at a liquid-gas or liquid-solid interphase. During the polymerization process, nano-fibres of CPs are created at first stage, however after a certain amount of growth, an excessive proliferation of nano-fibres can lead to an uneven scaffold of polymer matrix [69- 71]. Interfacial polymerization has the ability to restrain the excessive growth of CPs, as the polymerization of the fiber ceases once it exits the interface. This leads to the formation of high-quality polymer nanofibers [72, 73].
4.4 Photo Polymerization
In this process, the light irradiation is carried out on the monomer in order to start the polymerization process. Mostly, strong reducing chemicals are typically used to reduce GO in order to enhance its electrical characteristics. Strong reducing agents, on the other hand, reduce the effectiveness of GO by immediately contaminating the reducing agent. Therefore, utilising this technology, GO polymerization and reduction can be done simultaneously. A typical light source for polymerization is UV irradiation.
Three commonly documented approaches for fabricating hybrid electrodes of GP and P@Ani in the literature are as follows: chemical polymerization in situ facilitated by acid and ammonium persulfate, physical blending of GP and P@Ani nanofillers and electrochemical polymerization in situ at the anode [74]. Among all three methods, in situ chemical polymerization method is cheap and easy approach for the startups. It requires no extra cost-effective equipment in the lab; only chemicals and basic glassware are required for the synthesis. On the other hand, electro polymerization method is easy, precise and fast as compared to the in-situ polymerization but not cost effective.
5. GP based P@Ani/P@Py and MO composite electrodes for SCs
The synthesis and characterization of R@GO composites for use in SCs have advanced significantly in the past few decades. Herein, we summarize the key findings from several notable studies that have contributed to the advancement of this field. Among the MOs used for electrode materials, the manganese dioxide (MnO2) is the most utilised but its low power and @spc are caused by its weak conductivity (~105 -10-6 S/m). In order to achieve high @spc and superior rate capability, the @EC of the MnO2 electrode must be improved. Therefore, it is believed that hybrid electrode designs will enhance charge-storage capacity and conductivity. [75].
The primary causes of MnO2’s low capacitance utilisation and capacitance fading are its weak electro-conductivity and irreversible phase transformation. Gong et al. proposed a unique method based on MnO2 material to create a hierarchical, binder-free electrode for stable SCs with high @spc [76]. They produced the MnO2 self-standing electrode on nitrogen-doped GP and single wall carbon nanotubes self-standing film (NGCF) by electrochemical deposition. As a result, the produced MnO2/NGCF cathode's electrochemical performance was ~488 Fg-1 at ~1 Ag-1. After ~10,000 charge-discharge cycles, the built symmetric aqueous SC showed long-life stability, a high voltage of ~2.4 V, and an @ED of ~106.7 Wh kg-1 at ~1200 W kg-1.
The electrode's pH value has an impact on the performance as well. Mahdi et al. created symmetric SCs by creating MnO2/R@GO nanocomposite material [77]. The effect of the pH of the electrodeposition bath on the properties of the generated electrodes was considered. The findings demonstrated that the MnO2/RGO nanocomposite, at pH = 7 produced a maximum @spc of ~693 Fg−1 at a @CD of ~0.5 Ag−1. At current densities of ~0.5 and ~20 Ag−1, its @spcs were ~578 Fg−1 and ~290 Fg−1, respectively. At a @CD of ~4 Ag−1, the symmetric SC retained approximately ~79% of its initial capacitance after ~5000 consecutive cycles of charge and discharge. The manufactured SC obtained the highest @ED of about ~80 Wh kg−1 at the @PD of ~0.63 kW kg−1 and the highest @PD of ~25 kW kg−1 at the @ED of ~40 Wh kg−1.
One interesting option for lowering the total weight of electric vehicles is the structural SC. Sha and colleagues described a method to produce carbon fibre (CF) electrodes modified with vertical graphene (VG) and MnO2 in order to maximise the energy storage capacity of a load-carrying structure [78]. The outcomes demonstrated that there was a notable synergistic effect between the hybridization of VG and MnO2 in raising the electrode's areal capacitance. This cooperative effect was ascribed to VG's twin actions of boosting @EC and effective @SA, which allowed for improved MnO2 dispersion and a highly conductive network. Based on a polymer electrolyte and a CF/VG/MnO2 hybrid electrode, a structural SC demonstrated an areal capacitance of ~31 mF/cm2 and an @ED of ~12 mW h kg-1 and @PD of ~2210 mW kg-1.
In order to create a layer-by-layer (LbL) film for SC applications, Oliveira et al. incorporated MnO2 nanostructures into R@GO sheets and layered them with poly(allylamine-hydrochloride) (PAH) [79]. A SC with high areal capacitance of ~112 mF/cm2 (at ~1 mV/s) and ~460 Fg-1 (at ~1 Ag-1), as well as a high capacitive retention performance of ~99% over ~10,000 cycles and a high charge-discharge duration of ~600 s, is produced using nanostructured PAH/R@GO-MnO2 LbL films with ~20 bilayers.
Oliveira et al. integrated MnO2 nanostructures into R@GO sheets and stacked them with poly(allylamine-hydrochloride) (PAH) to produce a layer-by-layer (LbL) film for SC applications [79]. Nanostructured PAH/ R@GO-MnO2 LbL films with ~20 bilayers were used to create a SC with a high areal capacitance of ~112 mF/cm2 (at ~1 mV/s) and ~460 Fg-1 (at ~1 Ag-1), a high charge-discharge lifetime of ~600 s, and a high capacitive retention performance of ~99% over ~10,000 cycles.
A promising material for creating flexible micro-SCs is laser-induced graphene (LIG). The problem with this material is that its low conductivity and small @SA severely limit its performances. Reina et al. enhanced the performance of a LIG SC by using electrophoresis to decorate its surface: one electrode was painted with manganese oxide, while the other electrode was adorned with metal nitrides and metal carbides [80]. The completed gadget demonstrated a ~1.6 V operating voltage and a total capacitance of ~136 mFcm− 2. Moreover, the SC demonstrated stability over time, maintaining a ~99% efficiency after ~600 cycles of continuous current charge and discharge. The values of @ED and @PD were ~25.5 µWhcm− 2 and ~5 mWcm− 2, respectively.
In a related study reported by Rao et al., they created composite electrodes using LIG and MnO2 by hydrothermally processing the materials, which were then employed as electrodes in flexible SCs [81]. Since MnO2 may be deposited directly onto the LIG electrodes, this approach differs from conventional processes in that it does away with the need for binders for constructing composite electrodes. With its special LIG-MnO2 composite electrodes, the built all-Flexible SC demonstrated an impressive ~66.5 mF cm−2 areal capacitance at ~5 mV s−1 scan rate. At a @CD of ~0.2 mA cm−2, the device demonstrated an exceptionally high areal @ED (~2.3 mWh cm−2) and @PD (~19.7 mW cm−2). Additionally, after ~2000 cycles, an ~82% capacitance retention was noted and after ~100 bending cycles, an ~80% capacitance retention indicated that the device was flexible enough to withstand bending testing.
An ultrafine manganese oxide–carbon nanofiber composite was described by Yang et al.; which maintained a ~98% cycling durability for ~5000 cycles and provided a capacitance of up to ~179 Fg-1 [82]. The improved contact area between MnO2 and the carbon nanofibers produced positive synergistic effects for improved electrochemical performance.
R@GO, P@Ani and MnO2 trinary materials were synthesised in a single process by Wadekar et al. without the need for further oxidising or polymerizing agents or acidic conditions [83]. The material exhibited both the R@GO and P@Ani/MnO2 pseudo capacitance. The material showed excessive specific energy and specific power of ~66.6 Wh kg-1 and ~1800 W kg-1, respectively and the highest @spc of ~592 Fg-1 (at ~1 Ag-1 @CD).
An effective means to get high @ED is to widen the voltage window, although this is heavily dependent on the electrode material's design. Li et. al. reported the manufacturing of hierarchical GO, MnO2 and cobalt-nickel layered double hydroxide electrodes [84]. The built asymmetric SC had an @ED of ~82 W h kg−1 at a @PD of ~950 W kg−1 and a @spc of ~164 Fg-1 at ~1 Ag-1. In addition, the apparatus exhibited remarkable resistance to bending; after ~1000 bending cycles, the @spc maintained ~94.7% of its original value. Following ~10,000 cycles of charging and discharging, the gadget kept a ~92.6% capacity retention and nearly ~100% coulombic efficiency.
Rehman et al synthesized pristine Pr2O3, NiO, Co3O4 nanoparticles, binary P@ANI-Pr2O3, P@ANI-NiO, P@ANI-Co3O4, ternary Pr2O3–NiO–Co3O4 and quaternary P@ANI-Pr2O3–NiO–Co3O4 spherical core-shell nanocomposite [85]. They reported that the quaternary nanocomposite had a higher @spc (500 Fg-1) as compared to binary nanocomposites: ~134 Fg−1 (P@Ani-Pr2O3), ~143 Fg− 1 (P@Ani-Co3O4), ~256 Fg−1 (P@Ani-NiO) and P@Ani (~90.8 Fg−1) at a scan rate of ~5 m Vs−1. Moreover, the greatest @ED and @PD were observed in the quaternary nanocomposite. The P@Ani matrix, which promotes ion transport and the development of core-shell structure, which improves surface-dependent electrochemical characteristics, is the cause of the increased capacitance. Greater capacitance resulted from better surface-dependent electrochemical properties brought about by the creation of the core-shell structure and the promotion of ion transport by the P@Ani matrix.
Rajkumar et al synthesized FeCo2O4/P@Ani using the in-situ polymerization process and reported it as a good electrode material for electrolyte transfer, with easy accessibility for both electron and ion transfer [86]. At a @CD of ~1 Ag−1, the FeCo2O4/P@Ani electrode demonstrated a maximum specific capacity of ~940 C g−1. The outstanding kinetic reversibility and good cycling stability were revealed by the high-capacity retention (FeCo2O4/P@Ani) of ~94.5% after ~5000 GCD cycles at a @CD of ~1 Ag−1.
Shi et al. synthesised highly conductive and stable Ni-based metal organic framework (Ni-@MOF)/poly(3,4-ethylenedioxythiophene) (PEDOT) composites from Ni-@MOF nanosheets and 3,4-ethylenedioxythiophene (EDOT) monomer [87]. Electrochemical performance and conductivity are greatly enhanced when conducting PEDOT is covered on Ni-@MOF. The Ni-@MOF/PEDOT composites were reported to have an ultrahigh @spc of ~1401 Fg−1 and after ~1000 cycles at ~10 Ag−1, the @spc is retained for more than ~80% of the time. Additionally, an ASC built using activated carbon (AC) and Ni-@MOF/PEDOT is assembled, achieving a @PD of ~450 W kg−1 and an @ED of ~40 Wh kg−1.
Combining traditionally synthesised @MOF with other materials to build composite electrodes for high-performance SCs remains a difficulty due to its poor performance. Cao et al. created a range of NiCo-@MOF composite materials with varying concentrations of P@Py nanotubes (T-PPy), referred to as NiCo-@MOF/T-PPy-m (m = 2.5, 5, 10, 15 and 20) [88]. These materials were created by in situ formation of the bimetallic @MOF surrounding the PPy nanotubes. The NiCo-@MOF/T-PPy-10 composite (1:1 Ni/Co molar ratio, 10 mg of T-PPy), had the highest @spc at ~849 Cg−1 (at ~1 Ag−1), excellent cyclic stability and good performance rate. After ~10,000 cycles, the capacitance retention rate was ~90%. Additionally, the constructed asymmetric SC with active carbon as the negative electrode and NiCo-@MOF/T-PPy-10 as the positive electrode produced a high cyclic lifespan (~91% capacitance retention after ~10,000 cycles) and an exceptional @ED of ~58.4 Wh kg−1 at the @PD of ~747.6 W kg−1. The interpenetrating network structure made of T-PPy-interweaved NiCo-@MOF polyhedrons, resembling a "string of candied haws," was discovered to have the best capacitive performance in terms of increased conductivity of the resulting composite, improved dispersity of the bimetallic @MOF and more exposed active sites. Additionally, the interwoven structure improved the rate capability and cycling stability while reducing the stress change-induced structure or phase fluctuation brought on by repetitive volumetric swelling and shrinking during the charge/discharge cycle.
When nickel oxide (NiO) and R@GO were combined to create composites, they showed an increased @spc of ~171.3 Fg-1 at a @CD of ~5 Ag-1. In the presence of a ~6 mol/L KOH electrolyte, they also emphasised the reversibility of NiO as an electrode material [89]. The production of composites including P@Py, MnO2 and R@GO was described by Ates et al. Their research showed that when the active material, carbon black and polyvinyl alcohol (PVA) were combined with a working electrode in a mass ratio of 80:10:10, the maximum @spc of ~285.8 Fg-1 at a scan rate of ~1 mV/s was obtained [90].
The areal capacitance, specific energy and specific power of composites made of R@GO, P@Py and cobalt-iron oxide were enhanced by Ishaq et al.'s synthesis [91]. By using a hydrothermal technique, Kumar et al. were able to synthesise composites of NiO/Co3O4 and R@GO and achieve an amazing capacitance value of ~980 Fg-1 at ~1 Ag-1 when there was a PVA-KOH electrolyte present [92].
By adding P@Ani to the composite, Martins et al. were able to create composites of decreased GO, P@Ani/iron oxide and increased @spc of ~281 Fg-1 and good long-term stability of ~76% [93]. Zinc oxide (ZnO), polyols and R@GO were combined to create composites by Murali et al. [94]. When the composite was taken at a weight ratio of 2:1 (R@GO:ZnO), the researchers reported a maximum capacitance of ~6.4 Fg-1 against Ag/AgCl at a scan rate of 10 mV/s. In the presence of a 3 M KOH electrolyte, Saravanan et al. synthesised composites of R@GO, zinc oxide (ZnO) and poly(1,8-diaminonaphthalene) that showed a @spc value of 239 Fg-1 at a @CD of ~0.5 Ag-1 [95].
Zahra et al. achieved exceptional results for composites of R@GO and cerium aluminate (CeAlO3) synthesised by the hydrothermal technique, including a @spc of ~1442 Fg-1 at ~1 Ag-1 and an @ED of up to ~57 Wh kg-1 [96]. Using a hydrothermal technique, Imtiaz et al. synthesised composites of R@GO and magnesium indium oxide (MgIn2O4), achieving maximal @PD of ~260.3 W kg-1 in the presence of an aqueous ~2.0 M KOH electrolytic solution and @spc values ranging from ~1589 to ~452.5 Fg-1 at @CD ranging from ~1 to ~4 Ag-1 [97].
Direct growth of binder free NixMn1-xMoO4 on Ni foam was reported by Jabeen and colleagues, with a high specific capacity of ~1297 Cg-1 at ~1 Ag-1, suggesting that it could be a strong electrode material [98]. Furthermore, the addition of nickel may have improved structural stability, boosted redox activity, improved conductivity and facilitated ion diffusion. An @ED of ~152 Wh kg-1, a @PD of ~840 W kg-1 and an excellent retention of ~96.7 % after ~10,000 cycles were highlighted by the remarkable performance of the ASC.
The creation of high-performance energy storage devices is made possible by the many methodologies and notable breakthroughs in the synthesis of R@GO composites for SC applications, which are collectively demonstrated by these works.
6. Conclusions and perspectives
The synthesis and characterization of R@GO composites for supercapacitors (SCs) have seen remarkable advancements, addressing key challenges such as low conductivity and @spc. Notable progress has been made by integrating MnO2 with various conductive materials and exploring hybrid electrode designs, resulting in enhanced electrochemical performance. Combining MnO2 with nitrogen-doped graphene, carbon nanotubes and vertical graphene has significantly improved @EC and @spc. These hybrid electrodes demonstrate superior rate capabilities and high energy densities. Innovations in structural SCs for electric vehicles and flexible SCs have shown promising results. Methods such as electrophoretic deposition and hydrothermal processing have enabled the creation of flexible, binder-free electrodes with high areal capacitance and energy density.
Studies on the impact of electrodeposition bath pH and other synthesis conditions have revealed optimal parameters for achieving maximum @spc and long-term stability. The use of metal-organic frameworks (MOFs), polyaniline (P@Ani) and polypyrrole (P@Py) has led to composites with high @spc and excellent cycling stability. These materials facilitate ion transfer and enhance surface-dependent electrochemical properties. The development of novel composite materials, such as NiCo-MOF/T-P@Py, has demonstrated exceptional @spc, cyclic stability and energy density. These materials benefit from improved conductivity, better dispersity and more exposed active sites.
Expanding the voltage window through hierarchical electrode designs has achieved high energy densities, crucial for practical SC applications. The combination of various metal oxides with R@GO has proven effective in this regard.
In summary, the research and development in R@GO composites have significantly advanced the performance of SCs. The diverse strategies, including hybrid electrode designs, optimal synthesis conditions and innovative composite materials, have collectively pushed the boundaries of SC technology. These advancements pave the way for high-performance energy storage devices with broad applications in portable electronics, electric vehicles and beyond. Future research should continue to explore new material combinations and fabrication techniques to further enhance the capabilities of supercapacitors.
Acknowledgement
This research is supported financially by the Science & Engineering Research Board (SERB), a statutory body of the Department of Science & Technology, Government of India, under the Teachers Associateship for Research Excellence (TARE) Research Project Scheme (File No. TAR/2021/000128), awarded to Paramjit Singh, Gujranwala Guru Nanak Khalsa College, Civil Lines, Ludhiana- 141001, Punjab, India.
The authors wish to acknowledge their collaborators and peers for their invaluable insights that contributed to shaping this manuscript. Additionally, the authors extend their gratitude to the publishers for granting permission to reproduce figures used in this publication.
Conflict of Interest: Authors declare no conflict of interest.
References
[1]. Y. Shao, M. El-Kady, J. Sun, Y. Li, Q. Zhang, M. Zhu, H. Wang, B. Dunn, R. B. Kaner, "Design and Mechanisms of Asymmetric Supercapacitors", Chem. Rev., vol. 118, no., 18, pp. 9233-9280, 2018.
View Article
[2]. S. M. Alsufyani, R. Jafer, J. Iqbal, R. Alwafi, S. Bashir, S. Ramesh, K. Ramesh, "Carbon and polymer-based conducting platforms incorporated with electroactive metal-oxides/sulphides for energy storage devices", J. Energy Storage, vol. 84, part A, 110713, 2024.
View Article
[3]. P. Singh, R. Saini, R. Kumar, P. Kulriya, "Advanced Nanostructures Derived from Hybrid Composite of Polyaniline and rGO for High-Performance Supercapacitor Applications", J. Electron. Mater., 2024.
View Article
[4]. M. A. A. M. Abdah, N. H. N. Azman, S. Kulandaivalu, Y. Sulaiman, "Review of the use of transition-metal-oxide and conducting polymer-based fibres for high-performance supercapacitors", Materials & Design, vol. 186, pp. 108199, 2020.
View Article
[5]. Q. Wang, J. Yan, Z. Fan,"Carbon materials for high volumetric performance supercapacitors: design, progress, challenges and opportunities," Energy Environ. Sci.,vol. 09, no. 3, pp. 729-762, 2016.
View Article
[6]. S. Chen, L. Qiu, H. M. Cheng, "Carbon-Based Fibers for Advanced Electrochemical Energy Storage Devices," Chem. Rev., vol. 120, no. 5, pp. 2811-2878, 2020.
View Article
[7]. X. Wang, T. S. Mathis, K. Li, Z. Lin, L. Vlcek, T. Torita, N. C. Osti, C. Hatter, P. Urbankowski, A. Sarycheva, M. Tyagi, E. Mamontov, P. Simon, Y. Gogotsi, "Influences from solvents on charge storage in titanium carbide MXenes," Nature Energy, vol. 4, pp. 241-248, 2019.
View Article
[8]. B. Pal, S. Yang, S. Ramesh, V. Thangadurai, R. Jose, "Electrolyte selection for supercapacitive devices: a critical review," Nanoscale Adv., vol. 01, pp. 3807-3835, 2019.
View Article
[9]. Z. Zhai, L. Zhang, T. Du, B. Ren, Y. Xu, S. Wang, J. Miao, Z. Liu, "A review of carbon materials for supercapacitors," Materials & Design, vol. 221, pp. 111017, 2022.
View Article
[10]. C. An, Y. Zhang, H. Guo, Y. Wang, "Metal oxide-based supercapacitors: progress and Prospectives," Nanoscale Adv., vol. 01, pp. 4644-4658, 2019.
View Article
[11]. E. Dhandapani, S. Thangarasu, S. Ramesh, K. Ramesh, R. Vasudevan, N. Duraisamy, "Recent development and prospective of carbonaceous material, conducting polymer and their composite electrode materials for supercapacitor - A review," J. Energy Storage, vol. 52, part C, pp. 104937, 2022.
View Article
[12]. R. Saini, P. Singh, R. Kumar, P. Kulriya, S. Kumar, "Facile Synthesis of Polypyrrole/Reduced Graphene Oxide Composites for High-Performance Supercapacitor Applications", J. Electron. Mater., 2024. https://doi.org/10.1007/s11664-024-11193-8
View Article
[13]. S. Fleischmann, J. B. Mitchell,R. Wang, C. Zhan,D. Jiang, V. Presser, V. Augustyn, "Pseudocapacitance: From Fundamental Understanding to High Power Energy Storage Materials," Chem. Rev. vol. 120, no. 14., pp. 6738-6782, 2020.
View Article
[14]. P. Singh, "Composites Based on Conducting Polymers and Carbon Nanotubes for Supercapacitors", in Conducting Polymer Hybrids. Springer Series on Polymer and Composite Materials, V. Kumar, S. Kalia, H. Swart, Ed. Springer, Cham., 2017, pp. 305-336.
View Article
[15]. T. Brousse, D. Bélanger, J. W. Long, "To Be or Not To Be Pseudocapacitive," J. Electrochem. Soc., vol. 162, pp. A5185−A5189, 2015.
View Article
[16]. P. Simon, Y. Gogotsi, B. Dunn, "Where Do Batteries End and Supercapacitors Begin," Science, vol. 343, pp. 1210−1211, 2014.
View Article
[17]. V. Strauss, K. Marsh, M. D. Kowal, M. El-Kady, R. B. Kaner, "A Simple Route to Porous Graphene from Carbon Nanodots for Supercapacitor Applications," Adv. Mater., pp. 1704449, 2018.
View Article
[18]. P. Singh, R. Kumar, R. Prasad, "Free volume evolution in 50 MeV Li3+ ion-irradiated polymers studied by positron annihilation lifetime spectroscopy," Radiat. Eff. Defects Solids, vol. 168, no. 2, pp. 97-105, 2012.
View Article
[19]. P. Singh, R. Kumar, J. Cyriac, M.T. Rahul, P.M.G. Nambissan, R. Prasad, "High energy (MeV) ion fluence dependent nano scale free volume defects studies of PMMA films," Nucl. Instrum. Meth. B, vol. 320, pp. 64-69, 2014.
View Article
[20]. S. K. Gupta, R. Gupta, P. Singh, V. Kumar, M. K. Jaiswal, S.K. Chakarvarti, R. Kumar, "Modifications in physico-chemical properties of 100MeV oxygen ions irradiated polyimide Kapton-H polymer," Nucl. Instrum. Meth. B, vol. 406, part A, pp. 188-192, 2017.
View Article
[21]. R. Kumar, P. Singh, "Influence of SHI upon nanohole free volume and micro scale level surface modifications of polyethyleneterephthalate polymer films," Appl. Surf. Sci., vol. 337, pp. 19-26, 2015.
View Article
[22]. V. Kumar, R. Gupta, V. Chauhan, J. Ram, P. Singh, M. Prasad, R. Mehra, R. Kumar, "High-energy 120 MeV Au9+ ion beam-induced modifications and evaluation of craters in surface morphology of SnO2 and TiO2 nanocomposite thin films," Appl. Nanosci., Vol. 9, pp. 1265-1280, 2019.
View Article
[23]. J. G. Ibanez, M. E. Rincon, S. Gutierrez-Granados, M. Chahma, O. A. Jaramillo-Quintero, B. A. Frontana-Uribe, "Conducting Polymers in the Fields of Energy, Environmental Remediation and Chemical−Chiral Sensors," Chem. Rev., vol. 118, no. 9, pp. 4731-4816, 2018.
View Article
[24]. K. S. Ryu, K. M. Kim, N.G. Park, Y. J. Park, S. H. Chang, "Symmetric redox supercapacitor with conducting polyaniline electrodes," J. Power Sources, vol. 103, Issue 2, pp. 305-309, 2002.
View Article
[25]. K. S. Ryu, K. M. Kim, Y. J. Park, N. G. Park, M. G. Kang, S. H. Chang, "Redox supercapacitor using polyaniline doped with Li salt as electrode," Solid State Ionics," vol. 152-153, pp. 861-866, 2002.
View Article
[26]. M. Q. Wu, G. A. Snook, V. Gupta, M. Shaffer, D. J. Fray, G. Z. Chen, "Electrochemical fabrication and capacitance of composite films of carbon nanotubes and polyaniline," J. Mater. Chem., vol. 15, pp. 2297, 2005.
View Article
[27]. D. D. Zhou, X. T. Cui, A. Hines, R. J. Greenberg, "Conducting Polymers in Neural Stimulation Applications," in Implantable Neural Prostheses 2. Biological and Medical Physics, Biomedical Engineering, D. Zhou, E. Greenbaum, Ed. New York: Springer, NY, USA, 2009, pp. 217-252.
View Article
[28]. V. S. Jamadade, D. S. Dhawale, C. D. Lokhande, "Studies on electrosynthesized leucoemeraldine, emeraldine and pernigraniline forms of polyaniline films and their supercapacitive behavior," Synth. Metals vol. 160, pp. 955-960, 2010.
View Article
[29]. A. H. Majeed, L. A. Mohammed, O. G. Hammoodi, S. Sehgal, M. A. Alheety, K. K. Saxena, S. A. Dadoosh, I. K. Mohammed, M. M. Jasim, N. U. Salmaan, "A Review on Polyaniline: Synthesis, Properties, Nanocomposites and Electrochemical Applications," Int. J. Polym. Sci., vol. 2022, pp. 9047554, 2022.
View Article
[30]. M. Beygisangchin, S. A. Rashid, S. Shafie, A. R. Sadrolhosseini, H. N. Lim, "Preparations, Properties and Applications of Polyaniline and Polyaniline Thin Films- A Review," Polymers, vol. 13, pp. 2003, 2021.
View Article
[31]. N.V. Blinova, J. Stejskal, M. Trchova, J. Prokeš, "Control of polyaniline conductivity and contact angles by partial protonation," Polym. Int. vol. 57, pp. 66-69, 2008.
View Article
[32]. A. G. MacDiarmid, A. J. Epstein, "Faraday Discuss," Chem. Soc. vol. 88, pp. 317, 1989.
View Article
[33]. Z.A. Boeva, V.G. Sergeyev, "Polyaniline: Synthesis, properties and application," Polym. Sci. Ser. C, vol. 56, pp. 144-153, 2014.
View Article
[34]. G. A. Snook, P. Kao, A. S. Best, "Conducting-Polymer-Based Supercapacitor Devices and Electrodes," J. Power Sources, vol. 196, pp. 1−12, 2011.
View Article
[35]. N. K. Jangid, S. Jadoun, N. Kaur, "A review on high-throughput synthesis, deposition of thin films and properties of polyaniline," European Polym. J., vol. 125, pp. 109485, 2020.
View Article
[36]. Y. Cao, A. Andreatta, A. J. Heeger, P. Smith, "Influence of chemical polymerization conditions on the properties of polyaniline," Polym., vol. 30, no. 12, pp. 2305-2311, 1989.
View Article
[37]. H. Talbi, P.E. Just, L.H. Dao, "Electropolymerization of aniline on carbonized polyacrylonitrile aerogel electrodes: applications for supercapacitors," J. Appl. Electrochem. Vol. 33, pp. 465, 2003.
View Article
[38]. M. A. Deyab, G. Mele, E. Bloise, Q. Mohsen. "Novel nanocomposites of Ni-Pc/polyaniline for the corrosion safety of the aluminum current collector in the Li-ion battery electrolyte," Scientific Reports, vol. 11, no. 1, pp. 1-8, 2021.
View Article
[39]. A. K. Ghasemi, M. Ghorbani, M. S. Lashkenari, N. Nasiri, "Controllable synthesis of zinc ferrite nanostructure with tunable morphology on polyaniline nanocomposite for supercapacitor application," J. Energy Storage, vol. 51, pp. 104579, 2022.
View Article
[40]. A. A. Yaqoob, A. Serrà, S. A. Bhawani, M.N.M. Ibrahim, A. Khan, H.S. Alorfi, A.M. Asiri, M.A. Hussein, I. Khan, K. Umar, "Utilizing biomass-based graphene oxide-polyaniline-ag electrodes in microbial fuel cells to boost energy generation and heavy metal removal," Polymers, vol. 14, no. 4, pp. 845, 2022.
View Article
[41]. J. Upadhyay, T. M. Das, R. Borah, "Electrochemical performance study of polyaniline and polypyrrole based flexible electrodes," Inter. J. Polymer Analysis and Character., vol. 26, no. 4, 354-363, 2021.
View Article
[42]. C. H. Abdul Kadar, M. Faisal, N. Maruthi, N. Raghavendra, B. P. Prasanna, S. R. Manohara, "Corrosion-resistant polyaniline-coated zinc tungstate nanocomposites with enhanced electric properties for electromagnetic shielding applications," Macromolecular Research, vol. 30, pp. 638-649, 2022.
View Article
[43]. A. Samadi, M. Xie, J. Li, H. Shon, C. Zheng, S. Zhao, "Polyaniline-based adsorbents for aqueous pollutants removal: a review," Chem. Eng. J. vol. 418, pp. 129425, 2021.
View Article
[44]. X. Zhang, Y. Wang, D. Fu, G. Wang, H. Wei, N. Ma, "Photo-thermal converting polyaniline/ionic liquid inks for screen printing highly-sensitive flexible uncontacted thermal sensors," European Polym. J., vol. 147, pp. 110305, 2021.
View Article
[45]. G. P. Oliveira, B. H. Barboza, A. Batagin-Neto, "Polyaniline-based gas sensors: DFT study on the effect of side groups," Computational & Theoretical Chem., vol. 1207, pp. 113526, 2022.
View Article
[46]. Y. Mei, Z. Shen, S. Kundu, E. Dennis, S. Pang, F. Tan, G. Yue, Y. Gao, C. Dong, R. Liu, W. Zhang, M. I. Saidaminov, "Perovskite solar cells with polyaniline hole transport layers surpassing a 20% power conversion efficiency," Chem. Mater., vol. 33, no. 12, pp. 4679-4687, 2021.
View Article
[47]. S. Bhandari, "Polyaniline: Structure and Properties Relationship," in Polyaniline Blends, Composites and Nanocomposites, P.M. Visakh, C. D. Pina, E. Falletta, Ed. Elsevier, 2018, pp. 23-60.
View Article
[48]. A. L. Pang, A. Arsad, M. Ahmadipour, "Synthesis and factor affecting on the conductivity of polypyrrole: a short review," Polym. Adv. Technol., vol. 32, pp. 1428-1454, 2021.
View Article
[49]. L. J. Fang, L. H. Zhao, X. T. Liang, H. N. Xiao, L. Y. Qian, "Effects of oxidant and dopants on the properties of cellulose/PPy conductive composite hydrogels," J. Appl. Polym. Sci., vol. 133, no. 34, pp. 43759-43764, 2016.
View Article
[50]. K. Deshmukh, M. Basheer Ahamed, R. R. Deshmukh, S. K. Khadheer Pasha, P. R. Bhagat, K. Chidambaram, "Biopolymer composites with high dielectric performance: interface engineering," Biopolym. Compos. Electron., vol. 27, pp. 27-128, 2017.
View Article
[51]. M. Wolszczak, J. Kroh, M. M. Abdel-Hamid, "Some aspects of the radiation processing of conducting polymers," Radiat. Phys Chem., vol. 45, no. 1, pp. 71-78, 1995.
View Article
[52]. M. Rahaman, A. Aldalbahi, M. Almoiqli, S. Alzahly, "Chemical and electrochemical synthesis of polypyrrole using carrageenan as a dopant: polypyrrole/multi-walled carbon nanotube nanocomposites," Polymers, vol. 10, pp. 632-652, 2018.
View Article
[53]. S. Chavoshizadeh, S. Pirsa, F. Mohtarami, "Conducting/smart color film based on wheat gluten/chlorophyll/polypyrrole nanocomposite", Food Packag Shelf Life, vol. 24, pp. 100501-100511, 2020.
View Article
[54]. A. Mahun, S. Abbrent, P. Bober, J. Brus, L. Libor Kobera, "Effect of structural features of polypyrrole (PPy) on electrical conductivity reflected on 13CssNMR parameters," Synth. Met., vol. 259, pp. 116250- 111255, 2020.
View Article
[55]. T. H. Le, Y. Y. Kim, H. S. Yoon, "Electrical and electrochemical properties of conducting polymers," Polymers, vol. 9, pp. 150-181, 2017.
View Article
[56]. Y. Q. Cong, S. Z. Liu, H. F. Chen, "Fabrication of conductive polypyrrole nanofibers by electrospinning," J. Nanomater., vol. 2013, pp. 148347, 2013.
View Article
[57]. K. Lota, I. Acznik, A. Sierczynska, G. Lota, "Enhancing the performance of polypyrrole composites as electrode materials for supercapacitors by carbon nanotubes additives," J. Appl. Polym Sci., vol. 137, no. 28, pp. 48867-48874, 2019.
View Article
[58]. X. Y. Liu, L. Xue, Y. C. Lu, Y. J. Xia, Q. Li, "Fabrication of polypyrrole/multiwalled carbon nanotubes composites as high performance electrodes for supercapacitors," J. Electro Anal. Chem., vol. 862, pp. 114006- 114011, 2020.
View Article
[59]. E. M. Ryan, C. B. Breslin, "Formation of polypyrrole with dexamethasone as a dopant: its cation and anion exchange properties," J. Electro Anal. Chem., vol. 824, pp. 188-194, 2018.
View Article
[60]. Z. Zhang, Y. Zhang, K. Yang, K. Yi, Z. Zhou, A. Huang, K. Mai, X. Lu, "Three-dimensional carbon nanotube/ethylvinylacetate/polyaniline as a high performance electrode for supercapacitors," J. Mater. Chem. A, vol. 03, pp. 1884- 1889, 2015.
View Article
[61]. J. Li, H. Xie, Y. Li, J. Liu, Z. Li, "Electrochemical properties of graphene nanosheets/polyaniline nanofibers composites as electrode for supercapacitors," J. Power Sources, vol. 196, pp. 10775-10781, 2011.
View Article
[62]. D. D. Potphode, P. Sivaraman, S. P. Mishra, M. Patri, "Polyaniline/partially exfoliated multi-walled carbon nanotubes based nanocomposites for supercapacitors," Electrochim. Acta, vol. 155, pp. 402-410, 2015.
View Article
[63]. A.S. Arico, P. Bruce, B. Scrosati, J. M. Tarascon, W. Schalkwijk, "Nanostructured materials for advanced energy conversion and storage devices," Nat. Mater., vol. 04, pp. 366-377, 2005.
View Article
[64]. B. Wang, J. Qiu, H. Feng, E. Sakai, "Preparation of graphene oxide/polypyrrole/multi-walled carbon nanotube composite and its application in supercapacitors," Electrochim. Acta, vol. 151, pp. 230-239, 2015.
View Article
[65]. L. Yang, Z. Shi, W. Yang, "Polypyrrole directly bonded to air-plasma activated carbon nanotube as electrode materials for high-performance supercapacitor," Electrochim. Acta, vol. 153, pp. 76-82, 2015.
View Article
[66]. Y. Zhu, K. Shi, I. Zhitomirsky, "Polypyrrole coated carbon nanotubes for supercapacitor devices with enhanced electrochemical performance," J. Power Sources, vol. 268, pp. 233-239, 2014.
View Article
[67]. A. Singh, J. Dhau, R. Kumar, R. Badru, P. Singh, Y. K. Mishra, A. Kaushik, "Tailored carbon materials (TCM) for enhancing photocatalytic degradation of polyaromatic hydrocarbons," Progress in Materials Sci., vol. 144, pp. 101289, 2024.
View Article
[68]. T. A. Saleh, "Synthesis of hybrid materials: Methods and classification. Polymer hybrid materials and nanocomposites," William Andrew Publishing, pp. 177-212, 2021.
View Article
[69]. G. Ciric-Marjanovic, "Recent advances in polyaniline research: Polymerization mechanisms, structural aspects, properties and applications," Synth. Met., vol. 177, pp. 1-47, 2013.
View Article
[70]. J. Stejskal, I. Sapurina, M. Trchová, "Polyaniline nanostructures and the role of aniline oligomers in their formation," Prog. Polym. Sci., vol. 35, no. 12, pp. 1420-81, 2010.
View Article
[71]. L. Zhang, M. Wan, "Self-assembly of polyaniline-from nanotubes to hollow microspheres," Adv. Funct. Mater., vol. 13, no. 10, pp. 815-20, 2003.
View Article
[72]. J. Huang, R. B. Kaner, "A general chemical route to polyaniline nanofibers," J. Am Chem. Soc., vol. 126, no. 3, pp. 851-5, 2004.
View Article
[73]. J. Huang, R. B. Kaner, "The intrinsic nanofibrillar morphology of polyaniline," Chem. Commun., vol. 04, pp. 367-76, 2006.
View Article
[74]. Z. D. Huang, R. Liang, B. Zhang, Y. B. He, J. K. Kim, "Evolution of flexible 3D graphene oxide/ carbon nanotube/ polyaniline composite papers and their supercapacitive performance," Composites Sci. Technol., vol. 88, pp. 126-133, 2013.
View Article
[75]. M. Dai, D. Zhao, X. Wu, "Research progress on transition metal oxide-based electrode materials for asymmetric hybrid capacitors," Chinese Chem. Letters, vol. 31, no. 9, pp. 2177-2188, 2020.
View Article
[76]. D. Gong, H. Tong, J. Xiao, T. Li, J. Liu, Y. Wu, X. Chen, J. Liu, X. Zhang, "Self-standing manganese dioxide/graphene carbon nanotubes film electrode for symmetric supercapacitor with high energy density and superior long cycling stability," Ceramics Intern., vol. 47, no. 23, pp. 33020-33027, 2021.
View Article
[77]. F. Mahdi, M. Javanbakht, S. Shahrokhian, "In-site pulse electrodeposition of manganese dioxide/reduced graphene oxide nanocomposite for high-energy supercapacitors," J. Energy Storage, vol. 46, pp. 103802, 2022.
View Article
[78]. Z. Sha, F. Huang, Y. Zhou, J. Zhang, S. Wu, J. Chen, S. A. Brown, S. Peng, Z. Han, C. H. Wang, "Synergies of vertical graphene and manganese dioxide in enhancing the energy density of carbon fibre-based structural supercapacitors," Composites Sci. Technol., vol. 201, pp. 108568, 2021.
View Article
[79]. D. A. Oliveira, J. L. Lutkenhaus, J. R. Siqueira, "Building up nanostructured layer-by-layer films combining reduced graphene oxide-manganese dioxide nanocomposite in supercapacitor electrodes," Thin Solid Films, vol. 718, pp. 138483, 2021.
View Article
[80]. M. Reina, M. Serrapede, P. Zaccagnini, A. Pedico, M. Castellino, S. Bianco, T. Ouisse, H. Pazniak, J. Gonzalez-Julian, A. Lamberti, "Decoration of laser induced graphene with MXene and manganese oxide for fabrication of a hybrid supercapacitor," Electrochim. Acta, vol. 468, pp. 143163, 2023.
View Article
[81]. A. Rao, S. Bhat, S. De, "Binder-free laser induced graphene-MnO2 composite electrodes for high areal energy density flexible supercapacitors," Electrochim. Acta, vol. 487, pp. 144152, 2024.
View Article
[82]. Y. Yang, S. Lee, D.E. Brown, H. Zhao, X. Li, D. Jiang, S. Hao, Y. Zhao, D. Cong, X. Zhang, Y. Ren, "Fabrication of ultrafine manganese oxide-decorated carbon nanofibres for high-performance electrochemical capacitors," Electrochim. Acta, vol. 211, pp. 524-532, 2016.
View Article
[83]. P. H. Wadekar, R. V. Khose, D. A. Pethsangave, S. Some, "One-step Preparation of Conducting Polymer/Metal Oxide Doped RGO Trinary Composite for Supercapacitor Applications," ChemistrySelect, vol. 05, pp. 11769, 2020.
View Article
[84]. X. Li, Z. Lin, C. Wang, H. Wang, S. Feng, T. Li, Y. Ma, "Hierarchical graphene oxide/manganese dioxide/cobalt-nickel layered double hydroxide electrodes for high energy density asymmetric flexible supercapacitor," Chem. Engin. J., vol. 484, pp. 149430, 2024.
View Article
[85]. M. N. Rehman, T. Munawar, M. S. Nadeem, F. Mukhtar, A. Maqbool, M. Riaz, S. Manzoor, M. N. Ashiq, F. Iqbal, "Facile synthesis and characterization of conducting polymer-metal oxide based core-shell PANI-Pr2O-NiO-Co3O4 nanocomposite: As electrode material for supercapacitor," Ceramics International, vol. 47, no. 13, pp. 18497-18509, 2021.
View Article
[86]. S. Rajkumar, E. Elanthamilan, J. Princy Merlin, A. Sathiyan, "Enhanced electrochemical behaviour of FeCo2O4/PANI electrode material for supercapacitors," J. Alloys Compounds, vol. 874, pp. 159876, 2021.
View Article
[87]. L. Shi, W. Yang, X. Zha, Q. Zeng, D. Tu, Y. Li, Y. Yang, J. Xu, F. Chen, "In situ deposition of conducting polymer on metal organic frameworks for high performance hybrid supercapacitor electrode materials," J. Energy Storage, vol. 52, Part A, pp. 104729, 2022.
View Article
[88]. Y. Cao, N. Wu, F. Yang, M. Yang, T. Zhang, H. Guo, W. Yang, "Interpenetrating network structures assembled by "string of candied haws"-like PPY nanotube-interweaved NiCo-MOF-74 polyhedrons for high-performance supercapacitors," Colloids and Surfaces A: Physicochemical and Engineering Aspects, vol. 646, pp. 128954, 2022.
View Article
[89]. J. Xu, L. Wu, Y. Liu, J. Zhang, J. Liu, S. Shu, X. Kang, Q. Song, D. Liu, F. Huang, Y. Hu,"NiO-rGO composite for supercapacitor electrode," Surfaces and Interfaces, vol. 18, pp. 100420,2020.
View Article
[90]. M. Ates, I. Mizrak, O. Kuzgun, S. Aktas, "Synthesis, characterization and supercapacitor performances of activated and inactivated rGO/MnO2 and rGO/MnO2/PPy nanocomposites," Ionics, vol. 26, pp. 4723-4735, 2020.
View Article
[91]. S. Ishaq, M. Moussa, F. Kanwal, R. Ayub, T. N. Van, U. Azhar, D. Losic, "One step strategy for reduced graphene oxide/cobalt-iron oxide/polypyrrole nanocomposite preparation for high performance supercapacitor electrodes,"Electrochimica Acta, vol. 427, pp. 140883, 2022.
View Article
[92]. R. Kumar, R. Thangappan, F. Ran, S. Sambasivam, M. D. Albaqami, S. Mohammad,"Enriched performance of practical device assisted asymmetric supercapacitor: NiO/Co3O4 intercalated with rGO nanocomposite electrodes," J. Energy Storage, vol. 85, pp. 111075, 2024.
View Article
[93]. V. H. N. Martins, N. M. S. Siqueira, J. E. S. Fonsaca, S. H. Domingues, V. H. R. Souza, "Ternary Nanocomposites of Reduced Graphene Oxide, Polyaniline and Iron Oxide Applied for Energy Storage," ACS Appl. Nano Mater., vol. 4, no. 5, pp. 5553-5563, 2021.
View Article
[94]. S. Murali, P. K. Dammala, B. Rani, R. Santhosh, C. Jadhao, N. K. Sahu,"Polyol mediated synthesis of anisotropic ZnO nanomaterials and composite with rGO: Application towards hybrid supercapacitor," J. Alloys and Compounds, vol. 844, pp. 156149, 2020.
View Article
[95]. C. Saravanan, M. Karpuraranjith, K. Paramasivaganesh, P. M. Mareeswaran, A. Varghese, "Pseudocapacitive electrode performance of zinc oxide decorated reduced graphene oxide/poly(1,8-diaminonaphthalene) composite," J. Energy Storage, vol. 76, pp. 109792, 2024.
View Article
[96]. T. Zahra, B.M. Alotaibi, A. W. Alrowaily, H. A. Alyousef, M. F. Alotiby, H.H. Somaily, A.M.A. Henaish, "Facile hydrothermal synthesis of cerium aluminate (CeAlO3) and its composite with reduced graphene oxide (rGO) as an outstanding supercapacitor electrode," J. Energy Storage, vol. 87, pp. 111457, 2024.
View Article
[97]. M. Imtiaz, B.M. Alotaibi, A. W. Alrowaily, H. A. Alyousef, A. Dahshan, A.M.A. Henaish, "Fabrication of magnesium indium oxide (MgIn2O4) based on rGO for supercapacitor application," Diamond and Related Materials, vol. 144, pp. 111033, 2024.
View Article
[98]. S. Jabeen, P. Kumar, S. Sheokand, K. S. Samra,"Mesoporous Ni doped MnMoO4 nanoparticles for high performance asymmetric supercapacitors," J. Energy Storage, vol. 93, pp. 112464, 2024.
View Article
[1]. Y. Shao, M. El-Kady, J. Sun, Y. Li, Q. Zhang, M. Zhu, H. Wang, B. Dunn, R. B. Kaner, "Design and Mechanisms of Asymmetric Supercapacitors", Chem. Rev., vol. 118, no., 18, pp. 9233-9280, 2018. View Article
[2]. S. M. Alsufyani, R. Jafer, J. Iqbal, R. Alwafi, S. Bashir, S. Ramesh, K. Ramesh, "Carbon and polymer-based conducting platforms incorporated with electroactive metal-oxides/sulphides for energy storage devices", J. Energy Storage, vol. 84, part A, 110713, 2024. View Article
[3]. P. Singh, R. Saini, R. Kumar, P. Kulriya, "Advanced Nanostructures Derived from Hybrid Composite of Polyaniline and rGO for High-Performance Supercapacitor Applications", J. Electron. Mater., 2024. View Article
[4]. M. A. A. M. Abdah, N. H. N. Azman, S. Kulandaivalu, Y. Sulaiman, "Review of the use of transition-metal-oxide and conducting polymer-based fibres for high-performance supercapacitors", Materials & Design, vol. 186, pp. 108199, 2020. View Article
[5]. Q. Wang, J. Yan, Z. Fan,"Carbon materials for high volumetric performance supercapacitors: design, progress, challenges and opportunities," Energy Environ. Sci.,vol. 09, no. 3, pp. 729-762, 2016. View Article
[6]. S. Chen, L. Qiu, H. M. Cheng, "Carbon-Based Fibers for Advanced Electrochemical Energy Storage Devices," Chem. Rev., vol. 120, no. 5, pp. 2811-2878, 2020. View Article
[7]. X. Wang, T. S. Mathis, K. Li, Z. Lin, L. Vlcek, T. Torita, N. C. Osti, C. Hatter, P. Urbankowski, A. Sarycheva, M. Tyagi, E. Mamontov, P. Simon, Y. Gogotsi, "Influences from solvents on charge storage in titanium carbide MXenes," Nature Energy, vol. 4, pp. 241-248, 2019. View Article
[8]. B. Pal, S. Yang, S. Ramesh, V. Thangadurai, R. Jose, "Electrolyte selection for supercapacitive devices: a critical review," Nanoscale Adv., vol. 01, pp. 3807-3835, 2019. View Article
[9]. Z. Zhai, L. Zhang, T. Du, B. Ren, Y. Xu, S. Wang, J. Miao, Z. Liu, "A review of carbon materials for supercapacitors," Materials & Design, vol. 221, pp. 111017, 2022. View Article
[10]. C. An, Y. Zhang, H. Guo, Y. Wang, "Metal oxide-based supercapacitors: progress and Prospectives," Nanoscale Adv., vol. 01, pp. 4644-4658, 2019. View Article
[11]. E. Dhandapani, S. Thangarasu, S. Ramesh, K. Ramesh, R. Vasudevan, N. Duraisamy, "Recent development and prospective of carbonaceous material, conducting polymer and their composite electrode materials for supercapacitor - A review," J. Energy Storage, vol. 52, part C, pp. 104937, 2022. View Article
[12]. R. Saini, P. Singh, R. Kumar, P. Kulriya, S. Kumar, "Facile Synthesis of Polypyrrole/Reduced Graphene Oxide Composites for High-Performance Supercapacitor Applications", J. Electron. Mater., 2024. https://doi.org/10.1007/s11664-024-11193-8 View Article
[13]. S. Fleischmann, J. B. Mitchell,R. Wang, C. Zhan,D. Jiang, V. Presser, V. Augustyn, "Pseudocapacitance: From Fundamental Understanding to High Power Energy Storage Materials," Chem. Rev. vol. 120, no. 14., pp. 6738-6782, 2020. View Article
[14]. P. Singh, "Composites Based on Conducting Polymers and Carbon Nanotubes for Supercapacitors", in Conducting Polymer Hybrids. Springer Series on Polymer and Composite Materials, V. Kumar, S. Kalia, H. Swart, Ed. Springer, Cham., 2017, pp. 305-336. View Article
[15]. T. Brousse, D. Bélanger, J. W. Long, "To Be or Not To Be Pseudocapacitive," J. Electrochem. Soc., vol. 162, pp. A5185−A5189, 2015. View Article
[16]. P. Simon, Y. Gogotsi, B. Dunn, "Where Do Batteries End and Supercapacitors Begin," Science, vol. 343, pp. 1210−1211, 2014. View Article
[17]. V. Strauss, K. Marsh, M. D. Kowal, M. El-Kady, R. B. Kaner, "A Simple Route to Porous Graphene from Carbon Nanodots for Supercapacitor Applications," Adv. Mater., pp. 1704449, 2018. View Article
[18]. P. Singh, R. Kumar, R. Prasad, "Free volume evolution in 50 MeV Li3+ ion-irradiated polymers studied by positron annihilation lifetime spectroscopy," Radiat. Eff. Defects Solids, vol. 168, no. 2, pp. 97-105, 2012. View Article
[19]. P. Singh, R. Kumar, J. Cyriac, M.T. Rahul, P.M.G. Nambissan, R. Prasad, "High energy (MeV) ion fluence dependent nano scale free volume defects studies of PMMA films," Nucl. Instrum. Meth. B, vol. 320, pp. 64-69, 2014. View Article
[20]. S. K. Gupta, R. Gupta, P. Singh, V. Kumar, M. K. Jaiswal, S.K. Chakarvarti, R. Kumar, "Modifications in physico-chemical properties of 100MeV oxygen ions irradiated polyimide Kapton-H polymer," Nucl. Instrum. Meth. B, vol. 406, part A, pp. 188-192, 2017. View Article
[21]. R. Kumar, P. Singh, "Influence of SHI upon nanohole free volume and micro scale level surface modifications of polyethyleneterephthalate polymer films," Appl. Surf. Sci., vol. 337, pp. 19-26, 2015. View Article
[22]. V. Kumar, R. Gupta, V. Chauhan, J. Ram, P. Singh, M. Prasad, R. Mehra, R. Kumar, "High-energy 120 MeV Au9+ ion beam-induced modifications and evaluation of craters in surface morphology of SnO2 and TiO2 nanocomposite thin films," Appl. Nanosci., Vol. 9, pp. 1265-1280, 2019. View Article
[23]. J. G. Ibanez, M. E. Rincon, S. Gutierrez-Granados, M. Chahma, O. A. Jaramillo-Quintero, B. A. Frontana-Uribe, "Conducting Polymers in the Fields of Energy, Environmental Remediation and Chemical−Chiral Sensors," Chem. Rev., vol. 118, no. 9, pp. 4731-4816, 2018. View Article
[24]. K. S. Ryu, K. M. Kim, N.G. Park, Y. J. Park, S. H. Chang, "Symmetric redox supercapacitor with conducting polyaniline electrodes," J. Power Sources, vol. 103, Issue 2, pp. 305-309, 2002. View Article
[25]. K. S. Ryu, K. M. Kim, Y. J. Park, N. G. Park, M. G. Kang, S. H. Chang, "Redox supercapacitor using polyaniline doped with Li salt as electrode," Solid State Ionics," vol. 152-153, pp. 861-866, 2002. View Article
[26]. M. Q. Wu, G. A. Snook, V. Gupta, M. Shaffer, D. J. Fray, G. Z. Chen, "Electrochemical fabrication and capacitance of composite films of carbon nanotubes and polyaniline," J. Mater. Chem., vol. 15, pp. 2297, 2005. View Article
[27]. D. D. Zhou, X. T. Cui, A. Hines, R. J. Greenberg, "Conducting Polymers in Neural Stimulation Applications," in Implantable Neural Prostheses 2. Biological and Medical Physics, Biomedical Engineering, D. Zhou, E. Greenbaum, Ed. New York: Springer, NY, USA, 2009, pp. 217-252. View Article
[28]. V. S. Jamadade, D. S. Dhawale, C. D. Lokhande, "Studies on electrosynthesized leucoemeraldine, emeraldine and pernigraniline forms of polyaniline films and their supercapacitive behavior," Synth. Metals vol. 160, pp. 955-960, 2010. View Article
[29]. A. H. Majeed, L. A. Mohammed, O. G. Hammoodi, S. Sehgal, M. A. Alheety, K. K. Saxena, S. A. Dadoosh, I. K. Mohammed, M. M. Jasim, N. U. Salmaan, "A Review on Polyaniline: Synthesis, Properties, Nanocomposites and Electrochemical Applications," Int. J. Polym. Sci., vol. 2022, pp. 9047554, 2022. View Article
[30]. M. Beygisangchin, S. A. Rashid, S. Shafie, A. R. Sadrolhosseini, H. N. Lim, "Preparations, Properties and Applications of Polyaniline and Polyaniline Thin Films- A Review," Polymers, vol. 13, pp. 2003, 2021. View Article
[31]. N.V. Blinova, J. Stejskal, M. Trchova, J. Prokeš, "Control of polyaniline conductivity and contact angles by partial protonation," Polym. Int. vol. 57, pp. 66-69, 2008. View Article
[32]. A. G. MacDiarmid, A. J. Epstein, "Faraday Discuss," Chem. Soc. vol. 88, pp. 317, 1989. View Article
[33]. Z.A. Boeva, V.G. Sergeyev, "Polyaniline: Synthesis, properties and application," Polym. Sci. Ser. C, vol. 56, pp. 144-153, 2014. View Article
[34]. G. A. Snook, P. Kao, A. S. Best, "Conducting-Polymer-Based Supercapacitor Devices and Electrodes," J. Power Sources, vol. 196, pp. 1−12, 2011. View Article
[35]. N. K. Jangid, S. Jadoun, N. Kaur, "A review on high-throughput synthesis, deposition of thin films and properties of polyaniline," European Polym. J., vol. 125, pp. 109485, 2020. View Article
[36]. Y. Cao, A. Andreatta, A. J. Heeger, P. Smith, "Influence of chemical polymerization conditions on the properties of polyaniline," Polym., vol. 30, no. 12, pp. 2305-2311, 1989. View Article
[37]. H. Talbi, P.E. Just, L.H. Dao, "Electropolymerization of aniline on carbonized polyacrylonitrile aerogel electrodes: applications for supercapacitors," J. Appl. Electrochem. Vol. 33, pp. 465, 2003. View Article
[38]. M. A. Deyab, G. Mele, E. Bloise, Q. Mohsen. "Novel nanocomposites of Ni-Pc/polyaniline for the corrosion safety of the aluminum current collector in the Li-ion battery electrolyte," Scientific Reports, vol. 11, no. 1, pp. 1-8, 2021. View Article
[39]. A. K. Ghasemi, M. Ghorbani, M. S. Lashkenari, N. Nasiri, "Controllable synthesis of zinc ferrite nanostructure with tunable morphology on polyaniline nanocomposite for supercapacitor application," J. Energy Storage, vol. 51, pp. 104579, 2022. View Article
[40]. A. A. Yaqoob, A. Serrà, S. A. Bhawani, M.N.M. Ibrahim, A. Khan, H.S. Alorfi, A.M. Asiri, M.A. Hussein, I. Khan, K. Umar, "Utilizing biomass-based graphene oxide-polyaniline-ag electrodes in microbial fuel cells to boost energy generation and heavy metal removal," Polymers, vol. 14, no. 4, pp. 845, 2022. View Article
[41]. J. Upadhyay, T. M. Das, R. Borah, "Electrochemical performance study of polyaniline and polypyrrole based flexible electrodes," Inter. J. Polymer Analysis and Character., vol. 26, no. 4, 354-363, 2021. View Article
[42]. C. H. Abdul Kadar, M. Faisal, N. Maruthi, N. Raghavendra, B. P. Prasanna, S. R. Manohara, "Corrosion-resistant polyaniline-coated zinc tungstate nanocomposites with enhanced electric properties for electromagnetic shielding applications," Macromolecular Research, vol. 30, pp. 638-649, 2022. View Article
[43]. A. Samadi, M. Xie, J. Li, H. Shon, C. Zheng, S. Zhao, "Polyaniline-based adsorbents for aqueous pollutants removal: a review," Chem. Eng. J. vol. 418, pp. 129425, 2021. View Article
[44]. X. Zhang, Y. Wang, D. Fu, G. Wang, H. Wei, N. Ma, "Photo-thermal converting polyaniline/ionic liquid inks for screen printing highly-sensitive flexible uncontacted thermal sensors," European Polym. J., vol. 147, pp. 110305, 2021. View Article
[45]. G. P. Oliveira, B. H. Barboza, A. Batagin-Neto, "Polyaniline-based gas sensors: DFT study on the effect of side groups," Computational & Theoretical Chem., vol. 1207, pp. 113526, 2022. View Article
[46]. Y. Mei, Z. Shen, S. Kundu, E. Dennis, S. Pang, F. Tan, G. Yue, Y. Gao, C. Dong, R. Liu, W. Zhang, M. I. Saidaminov, "Perovskite solar cells with polyaniline hole transport layers surpassing a 20% power conversion efficiency," Chem. Mater., vol. 33, no. 12, pp. 4679-4687, 2021. View Article
[47]. S. Bhandari, "Polyaniline: Structure and Properties Relationship," in Polyaniline Blends, Composites and Nanocomposites, P.M. Visakh, C. D. Pina, E. Falletta, Ed. Elsevier, 2018, pp. 23-60. View Article
[48]. A. L. Pang, A. Arsad, M. Ahmadipour, "Synthesis and factor affecting on the conductivity of polypyrrole: a short review," Polym. Adv. Technol., vol. 32, pp. 1428-1454, 2021. View Article
[49]. L. J. Fang, L. H. Zhao, X. T. Liang, H. N. Xiao, L. Y. Qian, "Effects of oxidant and dopants on the properties of cellulose/PPy conductive composite hydrogels," J. Appl. Polym. Sci., vol. 133, no. 34, pp. 43759-43764, 2016. View Article
[50]. K. Deshmukh, M. Basheer Ahamed, R. R. Deshmukh, S. K. Khadheer Pasha, P. R. Bhagat, K. Chidambaram, "Biopolymer composites with high dielectric performance: interface engineering," Biopolym. Compos. Electron., vol. 27, pp. 27-128, 2017. View Article
[51]. M. Wolszczak, J. Kroh, M. M. Abdel-Hamid, "Some aspects of the radiation processing of conducting polymers," Radiat. Phys Chem., vol. 45, no. 1, pp. 71-78, 1995. View Article
[52]. M. Rahaman, A. Aldalbahi, M. Almoiqli, S. Alzahly, "Chemical and electrochemical synthesis of polypyrrole using carrageenan as a dopant: polypyrrole/multi-walled carbon nanotube nanocomposites," Polymers, vol. 10, pp. 632-652, 2018. View Article
[53]. S. Chavoshizadeh, S. Pirsa, F. Mohtarami, "Conducting/smart color film based on wheat gluten/chlorophyll/polypyrrole nanocomposite", Food Packag Shelf Life, vol. 24, pp. 100501-100511, 2020. View Article
[54]. A. Mahun, S. Abbrent, P. Bober, J. Brus, L. Libor Kobera, "Effect of structural features of polypyrrole (PPy) on electrical conductivity reflected on 13CssNMR parameters," Synth. Met., vol. 259, pp. 116250- 111255, 2020. View Article
[55]. T. H. Le, Y. Y. Kim, H. S. Yoon, "Electrical and electrochemical properties of conducting polymers," Polymers, vol. 9, pp. 150-181, 2017. View Article
[56]. Y. Q. Cong, S. Z. Liu, H. F. Chen, "Fabrication of conductive polypyrrole nanofibers by electrospinning," J. Nanomater., vol. 2013, pp. 148347, 2013. View Article
[57]. K. Lota, I. Acznik, A. Sierczynska, G. Lota, "Enhancing the performance of polypyrrole composites as electrode materials for supercapacitors by carbon nanotubes additives," J. Appl. Polym Sci., vol. 137, no. 28, pp. 48867-48874, 2019. View Article
[58]. X. Y. Liu, L. Xue, Y. C. Lu, Y. J. Xia, Q. Li, "Fabrication of polypyrrole/multiwalled carbon nanotubes composites as high performance electrodes for supercapacitors," J. Electro Anal. Chem., vol. 862, pp. 114006- 114011, 2020. View Article
[59]. E. M. Ryan, C. B. Breslin, "Formation of polypyrrole with dexamethasone as a dopant: its cation and anion exchange properties," J. Electro Anal. Chem., vol. 824, pp. 188-194, 2018. View Article
[60]. Z. Zhang, Y. Zhang, K. Yang, K. Yi, Z. Zhou, A. Huang, K. Mai, X. Lu, "Three-dimensional carbon nanotube/ethylvinylacetate/polyaniline as a high performance electrode for supercapacitors," J. Mater. Chem. A, vol. 03, pp. 1884- 1889, 2015. View Article
[61]. J. Li, H. Xie, Y. Li, J. Liu, Z. Li, "Electrochemical properties of graphene nanosheets/polyaniline nanofibers composites as electrode for supercapacitors," J. Power Sources, vol. 196, pp. 10775-10781, 2011. View Article
[62]. D. D. Potphode, P. Sivaraman, S. P. Mishra, M. Patri, "Polyaniline/partially exfoliated multi-walled carbon nanotubes based nanocomposites for supercapacitors," Electrochim. Acta, vol. 155, pp. 402-410, 2015. View Article
[63]. A.S. Arico, P. Bruce, B. Scrosati, J. M. Tarascon, W. Schalkwijk, "Nanostructured materials for advanced energy conversion and storage devices," Nat. Mater., vol. 04, pp. 366-377, 2005. View Article
[64]. B. Wang, J. Qiu, H. Feng, E. Sakai, "Preparation of graphene oxide/polypyrrole/multi-walled carbon nanotube composite and its application in supercapacitors," Electrochim. Acta, vol. 151, pp. 230-239, 2015. View Article
[65]. L. Yang, Z. Shi, W. Yang, "Polypyrrole directly bonded to air-plasma activated carbon nanotube as electrode materials for high-performance supercapacitor," Electrochim. Acta, vol. 153, pp. 76-82, 2015. View Article
[66]. Y. Zhu, K. Shi, I. Zhitomirsky, "Polypyrrole coated carbon nanotubes for supercapacitor devices with enhanced electrochemical performance," J. Power Sources, vol. 268, pp. 233-239, 2014. View Article
[67]. A. Singh, J. Dhau, R. Kumar, R. Badru, P. Singh, Y. K. Mishra, A. Kaushik, "Tailored carbon materials (TCM) for enhancing photocatalytic degradation of polyaromatic hydrocarbons," Progress in Materials Sci., vol. 144, pp. 101289, 2024. View Article
[68]. T. A. Saleh, "Synthesis of hybrid materials: Methods and classification. Polymer hybrid materials and nanocomposites," William Andrew Publishing, pp. 177-212, 2021. View Article
[69]. G. Ciric-Marjanovic, "Recent advances in polyaniline research: Polymerization mechanisms, structural aspects, properties and applications," Synth. Met., vol. 177, pp. 1-47, 2013. View Article
[70]. J. Stejskal, I. Sapurina, M. Trchová, "Polyaniline nanostructures and the role of aniline oligomers in their formation," Prog. Polym. Sci., vol. 35, no. 12, pp. 1420-81, 2010. View Article
[71]. L. Zhang, M. Wan, "Self-assembly of polyaniline-from nanotubes to hollow microspheres," Adv. Funct. Mater., vol. 13, no. 10, pp. 815-20, 2003. View Article
[72]. J. Huang, R. B. Kaner, "A general chemical route to polyaniline nanofibers," J. Am Chem. Soc., vol. 126, no. 3, pp. 851-5, 2004. View Article
[73]. J. Huang, R. B. Kaner, "The intrinsic nanofibrillar morphology of polyaniline," Chem. Commun., vol. 04, pp. 367-76, 2006. View Article
[74]. Z. D. Huang, R. Liang, B. Zhang, Y. B. He, J. K. Kim, "Evolution of flexible 3D graphene oxide/ carbon nanotube/ polyaniline composite papers and their supercapacitive performance," Composites Sci. Technol., vol. 88, pp. 126-133, 2013. View Article
[75]. M. Dai, D. Zhao, X. Wu, "Research progress on transition metal oxide-based electrode materials for asymmetric hybrid capacitors," Chinese Chem. Letters, vol. 31, no. 9, pp. 2177-2188, 2020. View Article
[76]. D. Gong, H. Tong, J. Xiao, T. Li, J. Liu, Y. Wu, X. Chen, J. Liu, X. Zhang, "Self-standing manganese dioxide/graphene carbon nanotubes film electrode for symmetric supercapacitor with high energy density and superior long cycling stability," Ceramics Intern., vol. 47, no. 23, pp. 33020-33027, 2021. View Article
[77]. F. Mahdi, M. Javanbakht, S. Shahrokhian, "In-site pulse electrodeposition of manganese dioxide/reduced graphene oxide nanocomposite for high-energy supercapacitors," J. Energy Storage, vol. 46, pp. 103802, 2022. View Article
[78]. Z. Sha, F. Huang, Y. Zhou, J. Zhang, S. Wu, J. Chen, S. A. Brown, S. Peng, Z. Han, C. H. Wang, "Synergies of vertical graphene and manganese dioxide in enhancing the energy density of carbon fibre-based structural supercapacitors," Composites Sci. Technol., vol. 201, pp. 108568, 2021. View Article
[79]. D. A. Oliveira, J. L. Lutkenhaus, J. R. Siqueira, "Building up nanostructured layer-by-layer films combining reduced graphene oxide-manganese dioxide nanocomposite in supercapacitor electrodes," Thin Solid Films, vol. 718, pp. 138483, 2021. View Article
[80]. M. Reina, M. Serrapede, P. Zaccagnini, A. Pedico, M. Castellino, S. Bianco, T. Ouisse, H. Pazniak, J. Gonzalez-Julian, A. Lamberti, "Decoration of laser induced graphene with MXene and manganese oxide for fabrication of a hybrid supercapacitor," Electrochim. Acta, vol. 468, pp. 143163, 2023. View Article
[81]. A. Rao, S. Bhat, S. De, "Binder-free laser induced graphene-MnO2 composite electrodes for high areal energy density flexible supercapacitors," Electrochim. Acta, vol. 487, pp. 144152, 2024. View Article
[82]. Y. Yang, S. Lee, D.E. Brown, H. Zhao, X. Li, D. Jiang, S. Hao, Y. Zhao, D. Cong, X. Zhang, Y. Ren, "Fabrication of ultrafine manganese oxide-decorated carbon nanofibres for high-performance electrochemical capacitors," Electrochim. Acta, vol. 211, pp. 524-532, 2016. View Article
[83]. P. H. Wadekar, R. V. Khose, D. A. Pethsangave, S. Some, "One-step Preparation of Conducting Polymer/Metal Oxide Doped RGO Trinary Composite for Supercapacitor Applications," ChemistrySelect, vol. 05, pp. 11769, 2020. View Article
[84]. X. Li, Z. Lin, C. Wang, H. Wang, S. Feng, T. Li, Y. Ma, "Hierarchical graphene oxide/manganese dioxide/cobalt-nickel layered double hydroxide electrodes for high energy density asymmetric flexible supercapacitor," Chem. Engin. J., vol. 484, pp. 149430, 2024. View Article
[85]. M. N. Rehman, T. Munawar, M. S. Nadeem, F. Mukhtar, A. Maqbool, M. Riaz, S. Manzoor, M. N. Ashiq, F. Iqbal, "Facile synthesis and characterization of conducting polymer-metal oxide based core-shell PANI-Pr2O-NiO-Co3O4 nanocomposite: As electrode material for supercapacitor," Ceramics International, vol. 47, no. 13, pp. 18497-18509, 2021. View Article
[86]. S. Rajkumar, E. Elanthamilan, J. Princy Merlin, A. Sathiyan, "Enhanced electrochemical behaviour of FeCo2O4/PANI electrode material for supercapacitors," J. Alloys Compounds, vol. 874, pp. 159876, 2021. View Article
[87]. L. Shi, W. Yang, X. Zha, Q. Zeng, D. Tu, Y. Li, Y. Yang, J. Xu, F. Chen, "In situ deposition of conducting polymer on metal organic frameworks for high performance hybrid supercapacitor electrode materials," J. Energy Storage, vol. 52, Part A, pp. 104729, 2022. View Article
[88]. Y. Cao, N. Wu, F. Yang, M. Yang, T. Zhang, H. Guo, W. Yang, "Interpenetrating network structures assembled by "string of candied haws"-like PPY nanotube-interweaved NiCo-MOF-74 polyhedrons for high-performance supercapacitors," Colloids and Surfaces A: Physicochemical and Engineering Aspects, vol. 646, pp. 128954, 2022. View Article
[89]. J. Xu, L. Wu, Y. Liu, J. Zhang, J. Liu, S. Shu, X. Kang, Q. Song, D. Liu, F. Huang, Y. Hu,"NiO-rGO composite for supercapacitor electrode," Surfaces and Interfaces, vol. 18, pp. 100420,2020. View Article
[90]. M. Ates, I. Mizrak, O. Kuzgun, S. Aktas, "Synthesis, characterization and supercapacitor performances of activated and inactivated rGO/MnO2 and rGO/MnO2/PPy nanocomposites," Ionics, vol. 26, pp. 4723-4735, 2020. View Article
[91]. S. Ishaq, M. Moussa, F. Kanwal, R. Ayub, T. N. Van, U. Azhar, D. Losic, "One step strategy for reduced graphene oxide/cobalt-iron oxide/polypyrrole nanocomposite preparation for high performance supercapacitor electrodes,"Electrochimica Acta, vol. 427, pp. 140883, 2022. View Article
[92]. R. Kumar, R. Thangappan, F. Ran, S. Sambasivam, M. D. Albaqami, S. Mohammad,"Enriched performance of practical device assisted asymmetric supercapacitor: NiO/Co3O4 intercalated with rGO nanocomposite electrodes," J. Energy Storage, vol. 85, pp. 111075, 2024. View Article
[93]. V. H. N. Martins, N. M. S. Siqueira, J. E. S. Fonsaca, S. H. Domingues, V. H. R. Souza, "Ternary Nanocomposites of Reduced Graphene Oxide, Polyaniline and Iron Oxide Applied for Energy Storage," ACS Appl. Nano Mater., vol. 4, no. 5, pp. 5553-5563, 2021. View Article
[94]. S. Murali, P. K. Dammala, B. Rani, R. Santhosh, C. Jadhao, N. K. Sahu,"Polyol mediated synthesis of anisotropic ZnO nanomaterials and composite with rGO: Application towards hybrid supercapacitor," J. Alloys and Compounds, vol. 844, pp. 156149, 2020. View Article
[95]. C. Saravanan, M. Karpuraranjith, K. Paramasivaganesh, P. M. Mareeswaran, A. Varghese, "Pseudocapacitive electrode performance of zinc oxide decorated reduced graphene oxide/poly(1,8-diaminonaphthalene) composite," J. Energy Storage, vol. 76, pp. 109792, 2024. View Article
[96]. T. Zahra, B.M. Alotaibi, A. W. Alrowaily, H. A. Alyousef, M. F. Alotiby, H.H. Somaily, A.M.A. Henaish, "Facile hydrothermal synthesis of cerium aluminate (CeAlO3) and its composite with reduced graphene oxide (rGO) as an outstanding supercapacitor electrode," J. Energy Storage, vol. 87, pp. 111457, 2024. View Article
[97]. M. Imtiaz, B.M. Alotaibi, A. W. Alrowaily, H. A. Alyousef, A. Dahshan, A.M.A. Henaish, "Fabrication of magnesium indium oxide (MgIn2O4) based on rGO for supercapacitor application," Diamond and Related Materials, vol. 144, pp. 111033, 2024. View Article
[98]. S. Jabeen, P. Kumar, S. Sheokand, K. S. Samra,"Mesoporous Ni doped MnMoO4 nanoparticles for high performance asymmetric supercapacitors," J. Energy Storage, vol. 93, pp. 112464, 2024. View Article